Doris Taylor doesn't take it as an insult when people call her Dr Frankenstein. “It was actually one of the bigger compliments I've gotten,” she says — an affirmation that her research is pushing the boundaries of the possible. Given the nature of her work as director of regenerative medicine research at the Texas Heart Institute in Houston, Taylor has to admit that the comparison is apt. She regularly harvests organs such as hearts and lungs from the newly dead, re-engineers them starting from the cells and attempts to bring them back to life in the hope that they might beat or breathe again in the living.
Taylor is in the vanguard of researchers looking to engineer entire new organs, to enable transplants without the risk of rejection by the recipient's immune system. The strategy is simple enough in principle. First remove all the cells from a dead organ — it does not even have to be from a human — then take the protein scaffold left behind and repopulate it with stem cells immunologically matched to the patient in need. Voilà! The crippling shortage of transplantable organs around the world is solved.
Free podcast
Brendan Maher and Takanori Takebe discuss the techniques being used to create hearts and livers for transplant.

In practice, however, the process is beset with tremendous challenges. Researchers have had some success with growing and transplanting hollow, relatively simple organs such as tracheas and bladders (see go.nature.com/zvuxed). But growing solid organs such as kidneys or lungs means getting dozens of cell types into exactly the right positions, and simultaneously growing complete networks of blood vessels to keep them alive. The new organs must be sterile, able to grow if the patient is young, and at least nominally able to repair themselves. Most importantly, they have to work — ideally, for a lifetime. The heart is the third most needed organ after the kidney and the liver, with a waiting list of about 3,500 in the United States alone, but it poses extra challenges for transplantation and bioengineering. The heart must beat constantly to pump some 7,000 litres of blood per day without a back-up. It has chambers and valves constructed from several different types of specialized muscle cells called cardiomyocytes. And donor hearts are rare, because they are often damaged by disease or resuscitation efforts, so a steady supply of bioengineered organs would be welcome.
Taylor, who led some of the first successful experiments to build rat hearts1, is optimistic about this ultimate challenge in tissue engineering. “I think it's eminently doable,” she says, quickly adding, “I don't think it's simple.” Some colleagues are less optimistic. Paolo Macchiarini, a thoracic surgeon and scientist at the Karolinska Institute in Stockholm, who has transplanted bioengineered tracheas into several patients, says that although tissue engineering could become routine for replacing tubular structures such as windpipes, arteries and oesophagi, he is “not confident that this will happen with more complex organs”.
Yet the effort may be worthwhile even if it fails, says Alejandro Soto-Gutiérrez, a researcher and surgeon at the University of Pittsburgh in Pennsylvania. “Besides the dream of making organs for transplantation, there are a lot of things we can learn from these systems,” he says — including a better basic understanding of cell organization in the heart and new ideas about how to fix one.
The scaffold
For more than a decade, biologists have been able to turn embryonic stem cells into beating heart-muscle cells in a dish. With a little electrical pacemaking from the outside, these engineered heart cells even fall into step and maintain synchronous beating for hours.
But getting from twitching blobs in a Petri dish to a working heart calls for a scaffold to organize the cells in three dimensions. Researchers may ultimately be able to create such structures with three-dimensional printing — as was demonstrated earlier this year with an artificial trachea2 (see Nature http://doi.org/m2q; 2013). For the foreseeable future, however, the complex structure of the human heart is beyond the reach of even the most sophisticated machines. This is particularly true for the intricate networks of capillaries that must supply the heart with oxygen and nutrients and remove waste products from deep within its tissues. “Vascularity is the major challenge,” says Anthony Atala, a urologist at Wake Forest University in Winston-Salem, North Carolina, who has implanted bioengineered bladders into patients3 and is working on building kidneys (see Naturehttp://doi.org/dw856h; 2006).
The leading techniques for would-be heart builders generally involve reusing what biology has already created. One good place to see how this is done is Massachusetts General Hospital in Boston, where Harald Ott, a surgeon and regenerative-medicine researcher, demonstrates a method that he developed while training under Taylor in the mid 2000s.
Suspended by plastic tubes in a drum-shaped chamber made of glass and plastic is a fresh human heart. Nearby is a pump that is quietly pushing detergent through a tube running into the heart's aorta. The flow forces the aortic valve closed and sends the detergent through the network of blood vessels that fed the muscle until its owner died a few days before. Over the course of about a week, explains Ott, this flow of detergent will strip away lipids, DNA, soluble proteins, sugars and almost all the other cellular material from the heart, leaving only a pale mesh of collagen, laminins and other structural proteins: the 'extracellular matrix' that once held the organ together.
The scaffold heart does not have to be human. Pigs are promising: they bear all the crucial components of the extracellular matrix, but are unlikely to carry human diseases. And their hearts are rarely weakened by illness or resuscitation efforts. “Pig tissues are much safer than humans and there's an unlimited supply,” says Stephen Badylak, a regenerative-medicine researcher at the University of Pittsburgh.
The tricky part, Ott says, is to make sure that the detergent dissolves just the right amount of material. Strip away too little, and the matrix might retain some of the cell-surface molecules that can lead to rejection by the recipient's immune system. Strip away too much, and it could lose vital proteins and growth factors that tell newly introduced cells where to adhere and how to behave. “If you can use a milder agent and a shorter time frame, you get more of a remodelling response,” says Thomas Gilbert, who studies decellularization at ACell, a company in Columbia, Maryland, that produces extracellular-matrix products for regenerative medicine.
Through trial and error, scaling up the concentration, timing and pressure of the detergents, researchers have refined the decellularization process on hundreds of hearts and other organs. It is probably the best-developed stage of the organ-generating enterprise, but it is only the first step. Next, the scaffold needs to be repopulated with human cells.
The cells
'Recellularization' introduces another slew of challenges, says Jason Wertheim, a surgeon at Northwestern University's Feinberg School of Medicine in Chicago, Illinois. “One, what cells do we use? Two, how many cells do we use? And three, should they be mature cells, embryonic stem cells, iPS [induced pluripotent stem] cells? What is the optimum cell source?”
Using mature cells is tricky to say the least, says Taylor. “You can't get adult cardiocytes to proliferate,” she says. “If you could, we wouldn't be having this conversation at all” — because damaged hearts could repair themselves and there would be no need for transplants.
Most researchers in the field use a mixture of two or more cell types, such as endothelial precursor cells to line blood vessels and muscle progenitors to seed the walls of the chambers. Ott has been deriving these from iPS cells — adult cells reprogrammed to an embryonic-stem-cell-like state using growth factors — because these can be taken from a patient in need and used to make immunologically matched tissues.
In principle, the iPS-cell approach could provide the new heart with its full suite of cell types, including vascular cells and several varieties of heart-muscle cell. But in practice, it runs into its own problems. One is the sheer size of a human heart. The numbers are seriously under-appreciated, says Ott. “It's one thing to make a million cells; another to make 100 million or 50 billion cells.” And researchers do not know whether the right cell types will grow when iPS cells are used to recapitulate embryonic development in an adult heart scaffold.
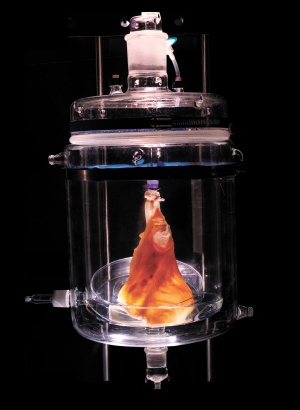
OTT LAB/MASSACHUSETTS GENERAL HOSPITAL
A decellularized human heart awaits rebuilding with an injection of precursor cells.
As they colonize the scaffold, some of the immature cells will take root and begin to grow. But urging them to become functional, beating cardiomyocytes requires more than just oxygenated media and growth factors. “Cells sense their environment,” says Angela Panoskaltsis-Mortari, who has been trying to build lungs for transplant at the University of Minnesota in Minneapolis. “They don't just sense the factors. They sense the stiffness and the mechanical stress,” which in turn pushes the cells down their proper developmental path.
So researchers must put the heart into a bioreactor that mimics the sensation of beating. Ott's bioreactors use a combination of electrical signals — akin to a pacemaker — to help to synchronize the beating cardiomyocytes seeded on the scaffold, combined with physical beating motions induced by a pump (see 'Customized organs'). But researchers face a constant battle in trying to ape the conditions present in the human body, such as changes in heart rate and blood pressure, or the presence of drugs. “The body reacts to things and changes the conditions so quickly it's probably impossible to mimic that in a bioreactor,” says Badylak.
When Taylor and Ott were first developing bioreactors, for decellullarized and repopulated rat hearts, they had to learn as they went along. “There was a lot of duct tape in the lab,” Ott says. But eventually the hearts were able to beat on their own after eight to ten days in the bioreactor, producing roughly 2% of the pumping capacity of a normal adult rat heart1. Taylor says that she has since got hearts from rats and larger mammals to pump with as much as 25% of normal capacity, although she has not yet published the data. She and Ott are confident that they are on the right path.
The beat
The final challenge is one of the hardest: placing a newly grown, engineered heart into a living animal, and keeping it beating for a long time.
The integrity of the vasculature is the first barrier. Any naked bit of matrix serves as a breeding ground for clots that could be fatal to the organ or the animal. “You're going to need a pretty intact endothelium lining every vessel or you're going to have clotting or leakage,” says Gilbert.
Ott has demonstrated that engineered organs can survive for a time. His group has transplanted a single bioengineered lung into a rat, showing that it could support gas exchange for the animal, but the airspace fairly quickly filled with fluids4. And an engineered rat-kidney transplant that Ott's group reported early this year survived without clotting, but had only minimal ability to filter urine, probably because the process had not produced enough of the cell types needed by the kidney5(see Nature http://doi.org/m2r; 2013). Ott's team and others have implanted reconstructed hearts into rats, generally in the neck, in the abdomen or alongside the animal's own heart. But although the researchers can feed the organs with blood and get them to beat for a while, none of the hearts has been able to support the blood-pumping function. The researchers need to show that a heart has much higher ability to function before they can transplant it into an animal bigger than a rat.
With the heart, says Badylak, “you have to start with something that can function pretty well” from the moment the transplant is in place. “You can't have something pumping just 1 or 2 or 5% of the ejection fraction of the normal heart and expect to make a difference,” he says, referring to a common measure of pumping efficiency. There is little room for error. “We're just taking baby steps,” says Panoskaltsis-Mortari. “We're where people were with heart transplant decades ago.”
The decellularization process being cultivated by Ott and others is already informing the development of improved tissue-based valves and other parts of the heart and other organs. A bioengineered valve, for example, may last longer than mechanical or dead-tissue valves because they have the potential to grow with a patient and repair themselves. And other organs may not need to be replaced entirely. “I'd be surprised if within the next 5–7 years you don't see the patient implanted with at least part of an artery, lobes of a lung, lobes of a liver,” says Badylak.
Taylor suspects that partial approaches could aid patients with severe heart defects such as hypoplastic left heart syndrome, in which half the heart is severely underdeveloped. Restoring the other half, “essentially forces you to build the majority of the things you need”, she says.
And these efforts could hold lessons for the development of cell therapies delivered to the heart. Researchers are learning, for example, how heart cells develop and function in three dimensions. In the future, partial scaffolds, either synthetic or from cadavers, could allow new cells to populate damaged areas of hearts and repair them like patches.
The jars of ghostly floating organs might seem like a gruesome echo of the Frankenstein story, but Taylor says her work is a labour of love. “There are some days that I go, 'Oh my god, what have I gotten into?' On the other hand, all it takes is a kid calling you, saying 'Can you help my mother?' and it makes it all worthwhile.”
- Nature 499, 20–22 ()